Points
- High precision clock comparisons of different atoms will contribute to a redefinition of the unit of time.
- The comparison of optical lattice clocks using ytterbium and strontium atoms has attained a new record uncertainty, while drastically reducing the required measurement time.
- Ultra-precise frequency comparisons will make atoms a sensitive detector for exploring new physics.
In the JST Strategic Basic Research Program, University of Tokyo professor Hidetoshi Katori, RIKEN foreign postdoctoral researcher Nils Nemitz and the “Katori Innovative Space-Time Project” at RIKEN demonstrate a comparison of different optical clocks with a dramatically reduced measurement time.
Atomic clocks provide what are probably the most accurate measurements in all of science. The next generation of clocks, now in development in laboratories all over the world, promises an error of less than a second in the 13.8 billion years (4.3 × 1017 seconds) age of the universe. Comparing clocks to this level of accuracy becomes a challenge in itself and can require months of measurement time. The research project therefore focusses on “optical lattice clocksNote 1)”, originally proposed by Professor Katori in 2001, and now reports the results of comparing the frequencies of two optical lattice clocks working with the elements ytterbium and strontium. They find the frequency ratio with an uncertainty of less than 5 parts in 1017, surpassing the limit set by the implementation of the “Système international” (SI) second itself, and improving on the record uncertainty for a comparison between clocks based on different atoms.
This achievement has been reported in “Nature Photonics”, published online on February 29th (British time).
With such ultra-precise comparisons of different atomic systems, the atoms themselves will act as highly sensitive detectors that may open new windows into effects that are not otherwise accessible, probing for Dark Matter or exploring the interactions of the recently discovered Higgs boson.
Background
The official unit of time in the SI system of units is defined based on an electronic resonance of the cesium atom. Such a definition does not depend on a prototype and can be accurately reproduced at any place or time.
Atomic clocks based on this or similar transition frequencies enable many modern technologies such as satellite navigation systems or high-speed cellular networks. They also provide the international time scales International Atomic Time (TAI) and Coordinated Universal Time (UTC) with a stability that corresponds to less than one second error in 30 million years, or one part in 1015.
Even greater accuracy is possible by moving from transitions in the microwave range around 10 GHz to the much higher frequencies of visible light near 500 THz. This has become a practical technology over the last decade with the development of optical frequency combs, a Nobel-prize winning technology that allows such frequencies to be measured precisely.
The next-generation clocks based on such optical transitions, and therefore often referred to as “optical clocks” now approach uncertainties of one part in 1018. But before the definition of the second can be updated to take advantage of this accuracy, the clocks need to demonstrate that their results are reproducible over time and between different implementations.
Optical clocks are implemented in two different ways. One is “single ion clocks” proposed by Hans Dehmelt, Nobel Physics Prize winner in 1989, and the other is “optical lattice clocks”, originally proposed by Professor Hidetoshi Katori in 2001. Single ion clocks require a long measurement time to reach their full accuracy because they measure the signal of only one atom. Optical lattice clocks have the advantage of allowing shorter measurements by probing a large number of atoms held in a trap formed by carefully controlled laser beams at a “magic” wavelengthNote 2).
The previous record uncertainty for the ratio of different clock transition frequencies is 5.2 × 10-17 for the comparison of clocks working with single ions of mercury and aluminum. While two optical lattice clocks, operating with the element strontium, were shown to agree to two parts in 1018, comparisons of clocks working with different atoms have not previously achieved an accuracy beyond that of single ion clocks.
Details of the Research
The frequency difference between two perfectly identical clocks is known in advance: It has to be zero. A comparison between different clocks, often given in terms of the ratio between the measured frequencies, determines an actual physical quantity. The value of this ratio can also be determined to an accuracy that is limited only by that of the clocks, not by the definition of the second. Since the result is a simple number, it can easily be transferred and compared between laboratories all over the world. (Fig.1)
The research project has added laser systems to one of the cryogenic optical lattice clocks to allow it to trap and probe the rare-earth element ytterbium. The new ytterbium clock was then carefully evaluated for possible frequency shifts. The resulting systematic uncertainty of 3.5 × 10-17 is almost ten times smaller than the best previous value for an ytterbium optical lattice clock, published by the American standards institute NIST in 2009.
The ytterbium/strontium frequency ratio was measured as 1.207 507 039 343 337 749 ± 0.000 000 000 000 000 055, more easily expressed as a relative uncertainty of 4.6 × 10-17. This surpasses the limit set on absolute frequency measurements by the SI second itself, which even the best cesium fountain clocks cannot determine to an uncertainty of 10-16. The measurement sets a new uncertainty record for the ratio of different clock transition frequencies, improving on the previous value of 5.2 × 10-17 found for the comparison of clocks working with single ions of mercury and aluminum. (Fig.2, Fig.3)
All atomic clocks are affected by a fundamental effect known as quantum projection noisenote 3), and require many repeated measurements to reach the desired uncertainty even if all other sources of instability are eliminated. A great advantage of optical lattice clocks is that by trapping a large number of atoms, many such measurements are performed in parallel.
The next limit to the clock stability then results from the frequency fluctuations of the laser probing the atoms. To suppress the effects of this, the experiments use the technique of “synchronous interrogation”: Atoms in both clocks are exposed to the clock laser for the exact same interval, so that identical laser noise creates identical frequency errors in each clock. This common error then does not contribute to the frequency ratio determined for each measurement, which are repeated every 1.5 s.
Of course synchronous interrogation can only provide an advantage if the frequency fluctuations are indeed shared between the probe lasers in both clocks. This presents a challenge in a comparison between clocks with different resonant wavelengths, such as 578 nm (yellow) for ytterbium and 698 nm (red) for strontium. The research project managed to overcome this problem by using infrared transfer lasers and an optical frequency comb to reference the ytterbium probe laser to the same ultra-stable reference resonator as the strontium laser.
This first application of synchronous interrogation to comparing different optical clocks reduces the required measurement time by a factor of four. Overall the stability of the clock comparison is characterized by a statistical uncertaintynote 4) that decreases with measurement time τ as σy(τ) = 4 × 10-16 (τ/s)-1/2, the best value reported so far for comparisons of clocks not based on the same atom. Compared to the mercury/aluminum single ion measurement, measurement times are reduced by a factor of 90, and a time of less than 150 s is sufficient to reach an overall uncertainty of the ytterbium/strontium ratio of 5 × 10-17. (Fig.2)
Future Plans
As the evaluation and control of frequency shifts in the ytterbium and strontium clocks continues to improve, the observed stability will allow clock comparisons to an accuracy of one part in 1018 with convenient measurement times of less than two days, where single-ion clocks would require multiple months. The results of such comparisons will build confidence in the next generation of clocks and generate the data required for a smooth transition to a new definition of the second.
Ultra-precise comparisons between optical clocks also open new windows for investigating effects that are not otherwise accessible. Measurements of absorption lines in remote interstellar gas clouds have hinted at a possible variation of the fundamental constants determining the physical laws of our universe. The resulting shifts of the atomic resonances may be detected by comparing clocks based on different atoms. It has recently been proposed that the normally undetectable presence of Dark Matter might also appear as a fluctuation or oscillation in the value of fundamental constants, with a time-scale as short as seconds. Another recent proposal makes use of the frequency shifts resulting from short-range interactions within the atom itself to probe the forces mediated by the newly discovered Higgs boson.
Future comparisons between optical lattice clocks may therefore use their exceptional accuracy and stability to turn the atoms into sensitive detectors to explore new physical effects.
Figures

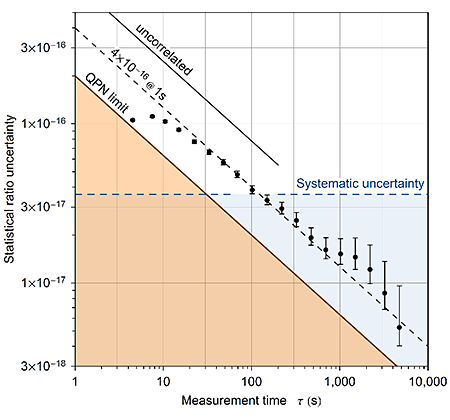
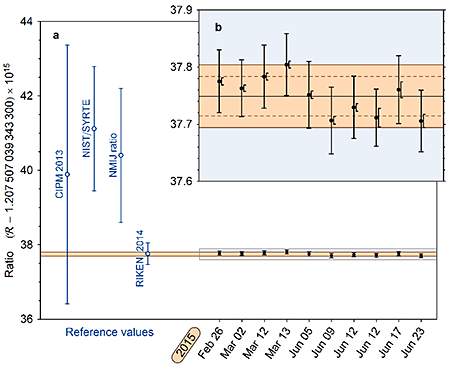
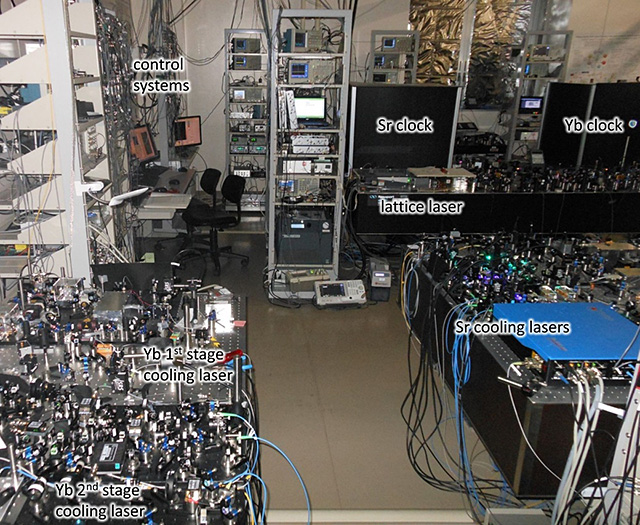
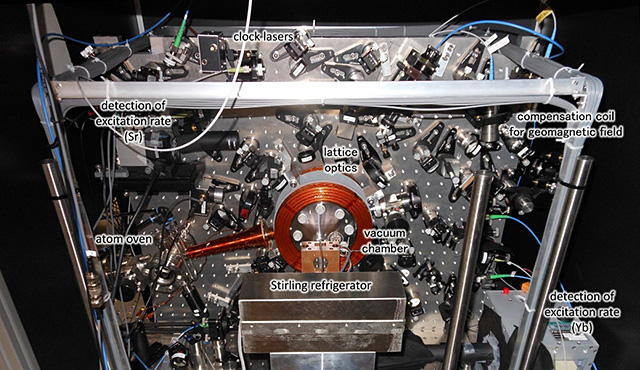
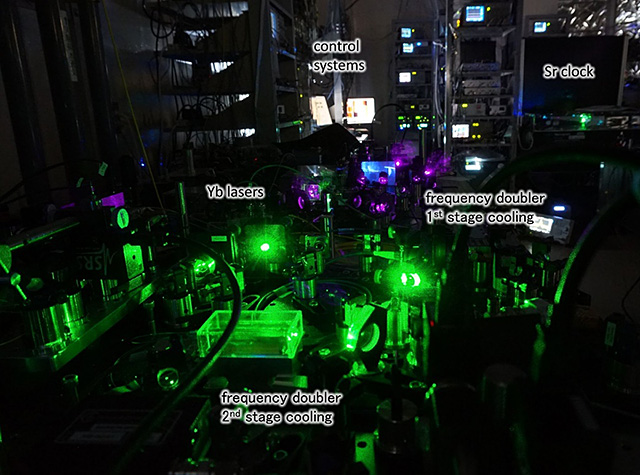
Technical Terms
- Note 1) Optical lattice clocks
- The optical lattice clock was originally proposed by Professor Hidetoshi Katori in 2001. The rapidly oscillating electric field of a laser beam can be used to trap neutral atoms, often in an “optical lattice” formed by the peaks of a standing wave. These peaks repeat at half the wavelength of the trapping laser (759 nm for ytterbium and 813 nm for strontium), offering many individual “sites” that atoms can be trapped in. As most sites contain no more than a single atom, interactions between atoms have very little effect on the measurement. The small dimension of the optical lattice plays another important role: Any motion of the atoms along the axis of the probe laser results in a shift of the observed resonance through the Doppler effect. But since the optical lattice restricts such motion on a scale shorter than the wavelength of the trapping and probing lasers, this shift is eliminated. In this way, the undisturbed resonance frequency of a large number of atoms can be probed simultaneously, for a precise measurement in a short measurement time.
- Note 2) Magic wavelength
- The same forces that create the trapping potential also affect the electronic energy levels and shift the resonance probed by the clock. The key discovery that enables optical lattice clocks is that the light used to generate the trap can be tuned to a magic wavelength, where the shifts for the two energy levels making up the clock transition become identical. The optical lattice then allows atoms to be trapped and strongly confined without introducing a frequencies error.
- Note 3) Quantum projection noise
- Optical clocks determine the atomic transition frequency by applying a probe laser and detecting if the atoms have been transferred to an excited atomic state or not. But while the probe laser leaves atoms in a superposition of (excited) and (not excited) states that varies smoothly with the frequency of the probe laser relative to the atomic resonance, the detection will “project” the state of each atom, and find it to either be excited or not excited. This is the origin of quantum projection noise, and is the equivalent of flipping a biased coin: The only way to determine the probability of flipping heads or tails is to repeat the flip many times and count the results.
- Note 4) Statistical uncertainty
- Effects like quantum projection noise and the frequency fluctuation of the probe laser introduce a random element into the outcome of each clock frequency measurement. The resulting statistical uncertainty can be reduced by repeating the measurement many times. A common way to describe this improvement with increasing measurement time τ is the Allan deviation σy(τ), which generally falls as
for an atomic clock, requiring a four times longer averaging time to reduce the statistical uncertainty by half.
Program Information
The Exploratory Research for Advanced Technology (ERATO)
“Katori Innovative Space-Time Project”
Journal Information
Nils Nemitz, Takuya Ohkubo, Masao Takamoto, Ichiro Ushijima, Manoj Das, Noriaki Ohmae, and Hidetoshi Katori. “Frequency ratio of Yb and Sr clocks with 5×10-17 uncertainty at 150 seconds averaging time”. Nature Photonics (2016), doi:10.1038/nphoton.2016.20.
Contact
[About Research]
Hidetoshi Katori, Ph.D.
Professor, Department of Applied Physics, Graduate School of Engineering, The University of Tokyo, Chief Scientist, Quantum Metrology Laboratory, Advanced Science Institute, RIKEN
E-mail:
URL: http://www.jst.go.jp/erato/katori/erato_en/index.html
[About Program]
Department of Research Project, JST
E-mail: